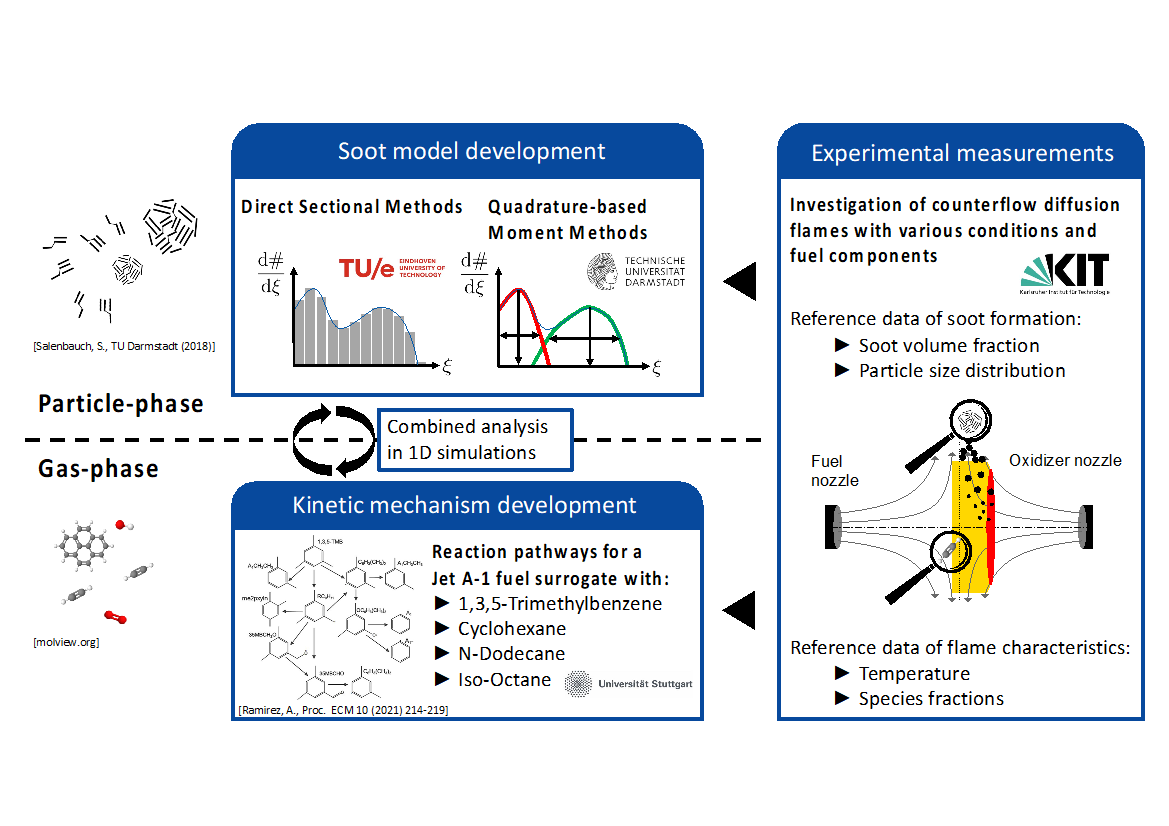
ESTiMatE’s researchers are synergistically working to combine the latest progress of chemical kinetics and soot model development as well as experimental measurements. A canonical flame configuration, the laminar counterflow diffusion flame, is employed for these studies. Here we explain the individual developments on the numerical models and experimental measurements and how the combination of these different approaches allows investigations of greater detail and ensures model validation.
Figure 1 illustrates the combined analysis strategy of the three areas of experimental measurements, modeling of the gas-phase chemistry and modeling of the particle-phase and gives an overview of interfaces between the individual fields which are described in more detail in the following.
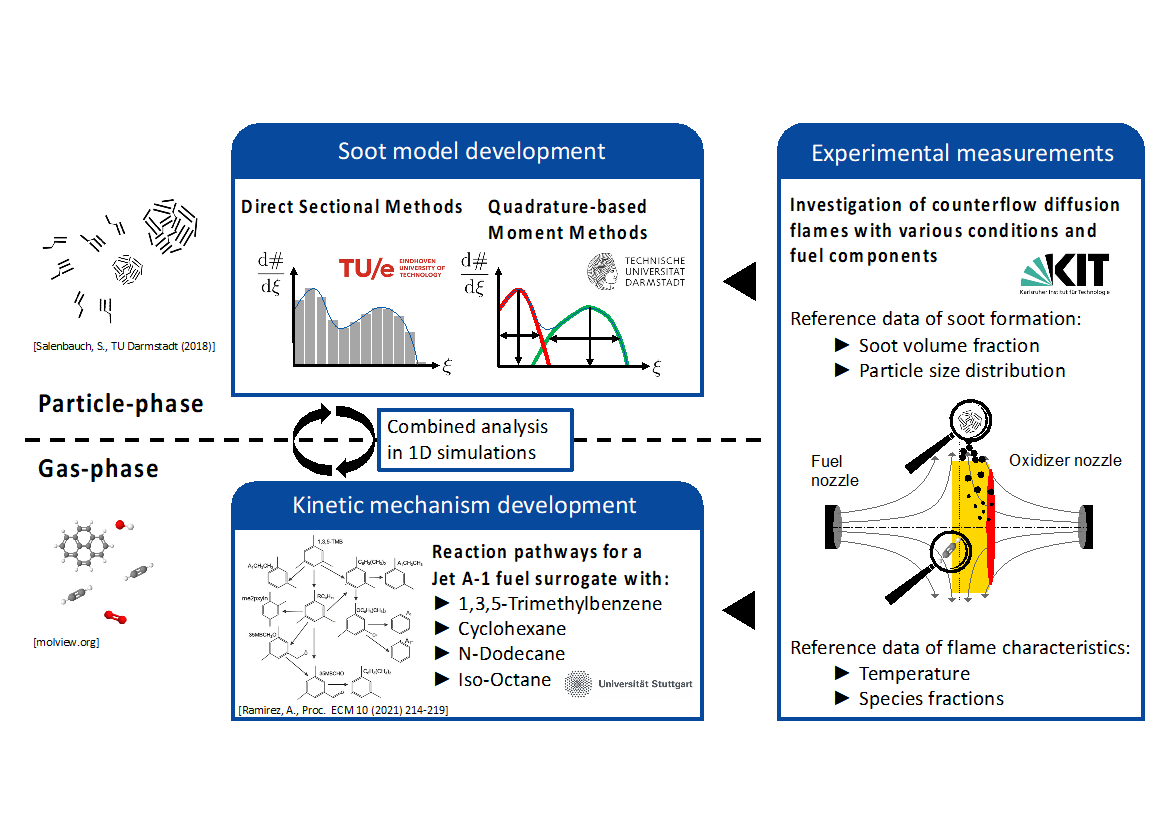
Figure 1: Combination and interaction of numerical modeling approaches with experimental measurements
Experimental Setup and Procedure
For the development and validation of chemical kinetic and soot models, experimental datasets gained under well-defined experimental conditions are of high importance. For this purpose, at the Engler-Bunte-Institute Chair of Combustion Technology at KIT experiments in non-premixed counterflow flames are performed. To address the aforementioned issue, non-premixed counterflow flames of different fuels like ethylene, iso-octane, surrogate A, etc., are investigated. The focus of these investigations is to determine the influence of different parameters like the fuel composition, fuels mass fraction, strain rate and pressure. The gained datasets include temperature profiles, species concentrations profiles and soot formation characteristics. For the temperature profile measurement, a coated thermocouple is used. Gaseous species concentrations profiles are measured by means of gas-chromatography and soot formation in the flames is determined by means of Laser-Induced Incandescence (LII).
The investigated non-premixed flames are stabilized on a counterflow burner under atmospheric pressure. A schematic illustration of the experimental setup can be seen in Figure 2. The fuel mixture is added to the system through the bottom duct and the oxidizer from the top. Further details about the design of the burner can be found here [1]. These investigations are performed under atmospheric pressure. The fuel stream was supplied in gaseous form, containing gaseous and pre-vaporized liquid components. The fuel line is heated up to 120°C (±15°C) to avoid condensation of the liquid components within the ducts or the burner. In all investigated flames, the oxidizer is air.
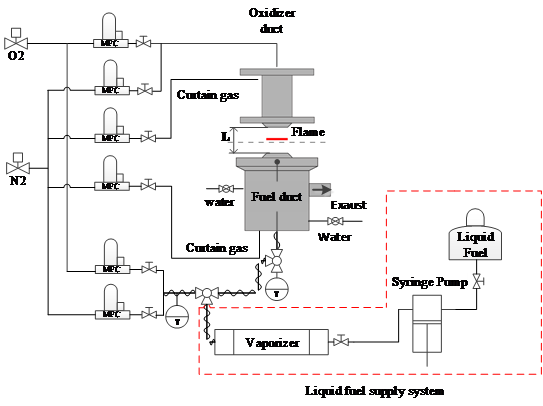
Figure 2: Schematic drawing of the counterflow burner experimental setup
The focus of this activity is to characterize the flame structure and soot formation of non-premixed counterflow flames of various fuels. The investigations started with ethylene, which is a gaseous fuel and widely investigated in the research combustion community. Afterwards, more relevant fuels for jet engine combustion, such as iso-octane and trimethylbenzene were investigated. Finally, we investigated the combustion properties of a jet surrogate fuel chosen in ESTiMatE (Surrogate A). Furthermore, the influence of fuel mass fraction and strain rate were investigated for all the fuels of interest.
The flame structure is investigated by measuring temperatures and analyzing major and minor species along the flame axis obtaining the profiles as a function of the distance from the fuel duct. Temperatures are measured with a fine wire S-type thermocouple (Pt10%Rh - Pt) with 100 μm diameter wires. The thermocouple used for these measurements was a constant tension thermocouple constructed in-house, following the design given by S. Ahuja and D. L. Miller [2]. Gas species concentrations in the examined flames are determined via gas chromatography using an in-situ probe sampling. The sampling system used in this study is similar to the one used in previous works of our group by A. Valencia-López et al. [1] that bases on the design presented by Sarathy [3] and Carbone et al. [4]. Downstream of the sampling system, the transfer line guiding the probe to the Gas Chromatograph is heated to 150°C to avoid condensation of species present in the flame probe. Further details about the Gas Chromatography system used for these investigations can be found in the work from M. Sentko et al. [5]. Soot formation is determined via Time-Resolved Laser-Induced Incandescence (TR-LII). In the experiments, the fundamental wavelength of a 10 Hz-pulsed Nd:YAG laser at 1064 nm (850 mJ/pulse) has been used. The time-resolved LII signal decays were collected at 120° angle to the laser beam using a lens system and two fast photomultipliers and the signals were recorded using a digital oscilloscope.
Development of a gas-phase kinetic mechanism for the description of a Jet A1 fuel oxidation kinetics and soot precursor formation
The numerical simulation of the combustion of a multi-component jet fuel commonly is performed by following the surrogate approach within the development of detailed chemical kinetic mechanisms. A surrogate is consisting of only a few components with well-known oxidation behavior and with its characteristics concerning thermo-chemical and physico-chemical properties being similar to those of Jet A-1 (fuel of interest in ESTiMatE). Considering the surrogate formulation, a chemical kinetic reaction mechanism is created starting from the reactants via the formation and decomposition of intermediate species up to the formation of the final combustion products. Such a model is usually built in a hierarchical way by describing the reaction network between all species existing within the oxidation of the fuel. Sub mechanisms for specific molecules must be incorporated to predict species of interest, for example, sub mechanisms for relevant species that act as soot precursors, such are PAHs (Polycyclic aromatic hydrocarbons).
Within ESTiMatE, a new reaction mechanism called ESTiMatE-Mech was generated by the group at the University of Stuttgart to describe the combustion of a Jet A-1 surrogate. Targeting its use in CFD modeling, first, a relevant 4 component surrogate for Jet A-1 was identified. Second, the mechanism consists of a limited number of species that will allow an efficient analysis and reduction process while keeping a good prediction of the most important combustion properties of the Jet A-1.
For the development of the ESTiMatE-Mech [6], a well-validated modular base mechanism was selected [7]. This base mechanism was modified in order to keep only those components necessary to model the Jet A-1 surrogate and new sub mechanisms were incorporated to account for the complete composition of the surrogate. The validation and optimization of the detailed reaction mechanism have been performed with the help of selected relevant validation data provided by the ESTiMatE partner KIT from laminar counter-flow diffusion flames.
While the kinetic mechanism describes the breakdown and oxidation of the fuel species and further kinetics in the gas-phase, particles are in a peri-condensed or solid form and considered by the soot model. The interface between the gas-phase and particle-phase is achieved by a transition from polycyclic aromatic hydrocarbons of the gas-phase to incipient soot particles in the solid-phase. To incorporate the influence of this two-way coupling, 1D simulations not only of the gas-phase but also with the coupled particle-phase are compared against experimental data.
Development of the soot formation and transition from gas-phase to particulate matter
Two groups (TU Eindhoven and TU Darmstadt) are working on the development of a soot formation model to describe the particulate emissions arising in aero-engine combustors.
To enable a detailed description of the various physical and chemical phenomena associated with soot formation, laminar counterflow flames measured at KIT are modeled with a discrete sectional method (DSM)-based soot model by the TU/e group. The current mono-variate sectional soot model [8] considers a discretized representation of the soot particle size distribution function (PSDF) in the particles’ volume space, and for each representative particle size (section), a transport equation for soot scalar (mass fraction) is solved. Furthermore, contributions of soot formation sub-processes such as nucleation, PAH-condensation, surface growth, oxidation, and coagulation are included in the soot model.
The group at TU Darmstadt is developing Quadrature-based Moment Methods (QbMM) [9] to describe the soot particle formation and evolution. In QbMM the PSDF is not solved directly but is approximated by solving only transport equations for its low-orders moments.
In the Split-based Extended Method of Moments (S-EQMOM) formulated in [10], schematically shown in Figure 1, the PSDF is reconstructed as the sum of sub-particle size distribution functions of a known shape, e.g. gamma functions, allowing a reduced number of transport equations needed to be solved. Therefore, the S-EQMOM provides a computationally efficient and robust local reconstruction of the PSDF.
The results of the soot model simulations are compared against experimentally evaluated soot volume fractions and particle size distributions. This enables, on one hand, a validation of the modeling strategy for the particulate matter and on the other hand through the availability of more quantities a deeper insight of the cause and effect chain leading to soot formation in these specific conditions burning Jet A-1 fuel.
References
[1] Valencia-López A. M. et al., Combustion and Flame, 207:265–80 (2019)
[2] Ahuja S., Miller D. L., Review of Scientific Instruments, 64:1358–9 (1993)
[3] Sarathy S. M., University of Toronto (2010)
[4] Carbone F., Gomez A., Combustion and Flame, 159:3040–55 (2019)
[5] Sentko M.M. et. al., Fuel, 286:119377 (2021).
[6] Ramirez-Hernandez, A.Y., Kathrotia, T., Methling, T., Braun-Unkhoff, M., Riedel, U. Gas Turbines Power GTP-21-1384. Accepted Manuscript
[7] Kathrotia, T., Oßwald, P., Naumann, C., Richter, S., Köhler, M., Fuel 302: 120736 (2021)
[8] Hoerlle, C. A., & Pereira, F. M. Combustion and Flame, 203:407-423 (2019)
[9] McGraw, R., Aerosol Sci. Technol. 27 (1997)
[10] Salenbauch, S. et al., J. of Aerosol Science 128, 34-49 (2019)