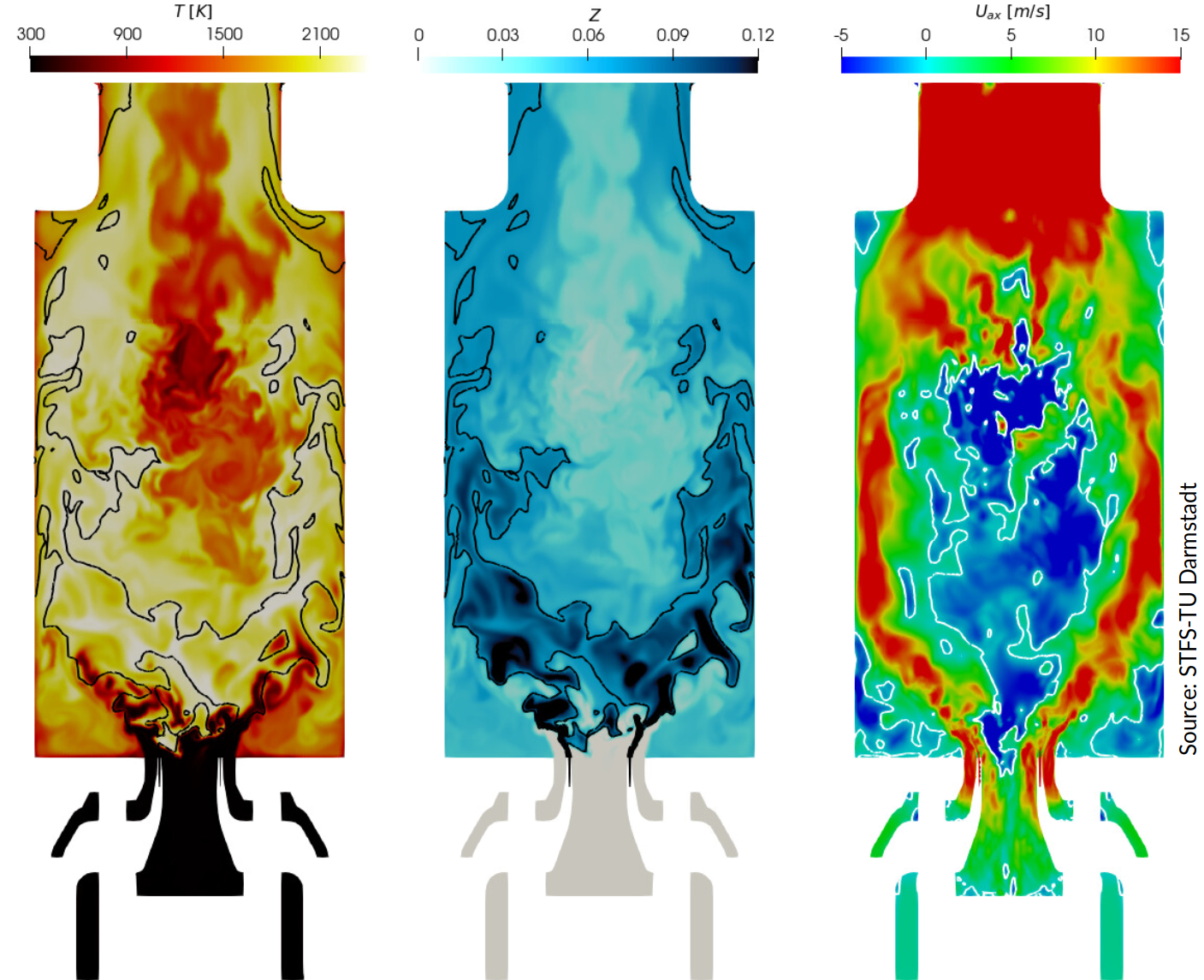
To improve thermal efficiencies, modern aircraft combustors are designed to operate at high combustion temperatures and elevated pressures. However, they will suffer from pollution emissions, such as unburnt hydrocarbons, nitrogen oxides, and soot. Soot emissions are especially harmful to human health and significantly contribute to global warming. Reducing particle emissions in future aero-engine combustors requires predictive and accurate soot models, together with turbulence and chemistry models.
The ESTiMatE project, which is part of the Clean Sky program, is focused on the development of advanced soot models to gain deeper knowledge in soot formation at relevant conditions of the aero engines.
In this context, the accurate prediction of the turbulent gas-phase chemical kinetics is the first essential component for soot modelling. The gas-phase soot precursors, polycyclic aromatic hydrocarbons (PAHs), need to be accounted for to characterise the particle formation and growth. The second fundamental component is the statistical model for the soot particle evolution, as an individual track of soot particles is computationally unrealistic. A valuable approach is developed and applied by Technical University Darmstadt (TUDa) and integrated into the Computational Fluid Dynamics (CFD) code OpenFOAM. The properties of the particles are described by a number density function (NDF), whose evolution is tracked by a population balance equation (PBE) depending on the turbulent thermophysical state. The PBE is not directly solved for, but only a small set of its low-order statistical moments using the Quadrature Method of Moments (QMOM) [1-2]. Herein, the NDF is reconstructed by a weighted sum of Dirac functions for QMOM or by a weighted sum of continuous distribution functions for the Extended Quadrature Method of Moments (EQMOM). This approach allows a detailed description of the particle formation and evolution in the flame at reasonable computational costs.
ESTiMatE researchers at TUDa are performing numerical simulations of a model aero-engine combustor using this approach. The investigated model combustor features flow field and flame conditions typical of a Rich-Burn/Quick-Quench/Lean-Burn (RQL) aero-engine combustor. Primary air is supplied to the flame through a central and annular nozzle. The air flows are separated and pass radial swirlers accounting for recirculation with a turbulent rich primary combustion zone. Gaseous fuel (C2H4) is injected between both airflows. Secondary air is injected into the chamber at a height of 80 mm leading to a RQL-type soot oxidation region. Experimental measurements performed by Geigle et al. [3–5] are available for the velocity field in both cold and reacting conditions as well as temperature and soot volume fraction data for several operating conditions (variations in pressure up to a maximum of 5 bar, equivalence ratio, and cases with and without oxidation air).
Instantaneous flow field variables in a midplane of the combustor. Left: Temperature, middle: Mixture fraction, right: Axial velocity. Black line: Stoichiometric mixture fraction, white line: Zero axial velocity
Large-Eddy Simulations have been performed with the OpenFOAM solver, extended at the TUDa with an in-house package for the solution of turbulent reacting flows using the flamelet-progress variable approach [6]. The artificial thickening approach (ATF) is used for the turbulence-chemistry interaction closure. Predictions of the velocity evolution and the reaction zone are adequately captured by the model when compared with experimental measurements. The QMOM soot model will be used to study the formation of soot particles within this combustion chamber, which will help researchers to understand the complex physics of soot formation guiding the path for the next generation of aero engines with fewer pollutant emissions.
[1] S. Salenbauch, C. Hasse, M. Vanni and D. L. Marchisio. A numerically robust method of moments with number density function reconstruction and its application to soot formation, growth and oxidation. Journal of Aerosol Science, 128, pp. 34-49, 2019.
[2] F. Ferraro, C. Russo. R. Schmitz, C. Hasse and M. Sirignano. Experimental and numerical study on the effect of oxymethylene ether-3 (OME3) on soot particle formation. Fuel, 286 (1), p. 119353, 2021.
[3] K.P Geigle, R. Hadef, M. Stöhr, and W. Meier. Flow field characterization of pressurized sooting swirl flames and relation to soot distributions. Proceedings of the Combustion Institute, 36(3):3917–3924, 2017.
[4] K.P Geigle, M. Köhler, W. O’Loughlin, and W. Meier. Investigation of soot formation in pressurized swirl flames by laser measurements of temperature, flame structures and soot concentrations. Proceedings of the Combustion Institute, 35(3):3373–3380, 2015.
[5] K.P. Geigle, W. O’Loughlin, R. Hadef, and W. Meier. Visualization of soot inception in turbulent pressurized flames by simultaneous measurement of laser-induced fluorescence of polycyclic aromatic hydrocarbons and laser-induced incandescence, and correlation to OH distributions. Applied Physics B: Lasers and Optics, 119(4):717–730, 2015.
[6] S. Popp, F. Hunger, S. Hartl, D. Messig, B. Coriton, J. H. Frank, F. Fuest, and C. Hasse, “LES flamelet-progress variable modeling and measurements of a turbulent partially-premixed dimethyl ether jet flame,” Combust. Flame, vol. 162, no. 8, pp. 3016–3029, Aug. 2015.